In recent years, the orthopedic device manufacturing industry has leveraged rapid prototyping technology to produce patient-specific implants. Most notably, additive manufacturing methods have been increasingly utilized to produce custom metal or PEEK implants for hip arthroplasty and spinal surgery. Metal additive technology has been advantageous by allowing quicker design iterations, minimizing weight via topology optimization, and allowing customizable implants to contour to a patient’s anatomy—with improved osseointegration as the primary objective.
Osseointegration, a key term in this article, is defined as the ability of an artificial implant to fuse with bone on a molecular level, improving functionality, strength, patient mobility, and safety.
A few design considerations that affect osseointegration may include, but are not limited to:
- Materials that limit or eliminate the possibility of cytotoxic molecules to be discharged from the implant (for example, cobalt ions discharged from a Cobalt-Chrome hip implant)
- Rough, porous osseointegrative surface quality where the part touches bone, allowing higher contact ratio and improved ‘bone anchorage’
- Part geometry (and material) that creates uniform load distribution, avoiding isolated load on a particular area of the part and/or bone over time that can cause part failure
However, osseointegrative surfaces and unique part designs are increasingly difficult to implement as demand for metal-AM grows and the limitations of the technology become increasingly apparent. As Metal-AM transitions into volume production for orthopedic implants, costs-per-part become critical, and manufacturers must seek to reduce these costs in creative ways—sometimes at the detriment of surface quality and part resolution. For example, manufacturers may reduce costs via larger powders, faster laser scan strategies, thicker layer lines, or more aggressive re-coater methods. These issues are exacerbated by the strict tolerance requirements and superfinished surface quality needed for implants to have sufficient fatigue strength, biocompatibility, and osseointegration.
Fortunately, there may be a technology capable of alleviating some of these manufacturing challenges for high-volume AM parts. Pulsed electrochemical machining, improves part resolution and surface quality for metal orthopedic implants in higher part volumes.
How PECM Works
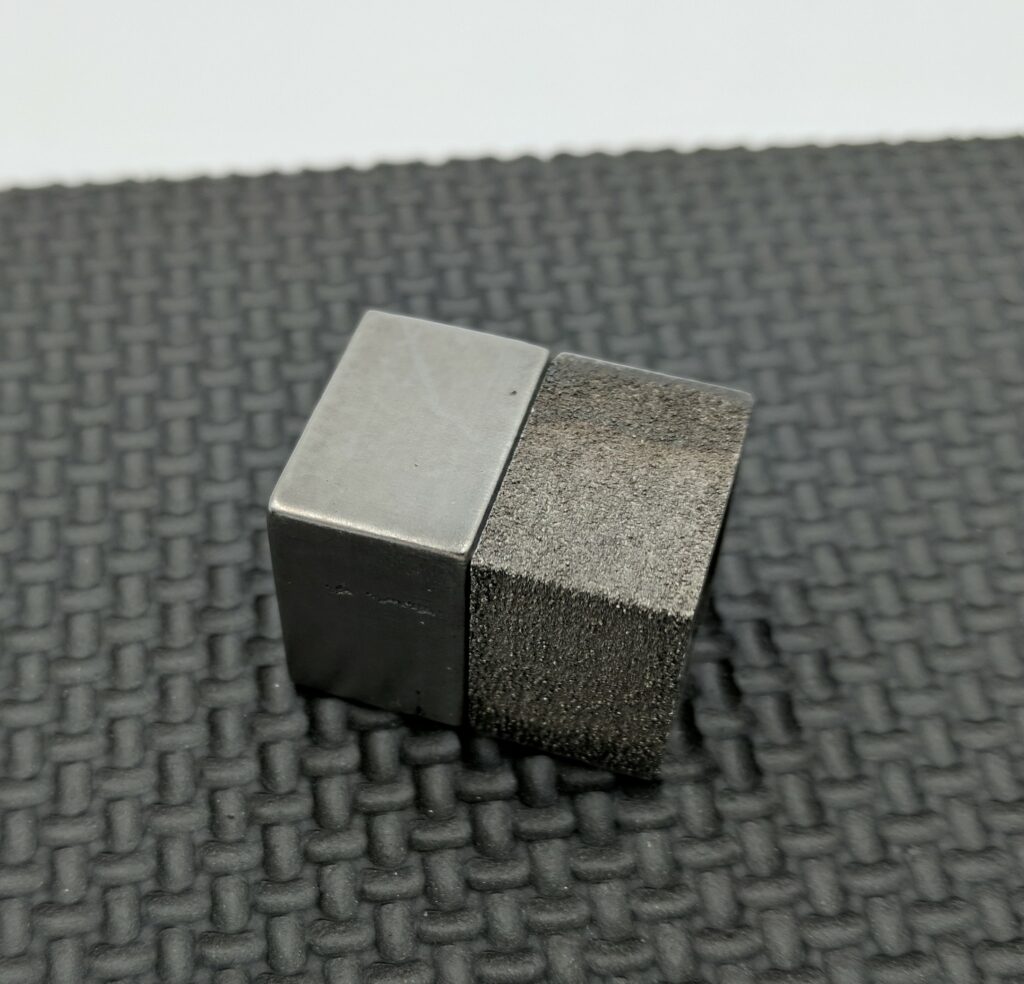
PECM is a non-contact, non-thermal material removal process that utilizes electrochemistry, as opposed to friction and/or heat, to remove the workpiece material. PECM flushes a charged electrolytic fluid within a microscopic gap between the tool (cathode) and workpiece (anode), and the proximity of the tool and workpiece is correlated with the removal rate of the workpiece. To do so, the cathode is shaped as the inverse of the desired geometry on the workpiece.
This electrolytic fluid simultaneously performs two crucial functions. First, it acts as the conductor for the electrochemical reaction to take place. Secondly, the fluid acts as a flushing agent that removes the waste products leftover from the reaction, and eliminates any residual heat that may otherwise affect the local conductivity of the electrolyte.
The unique nature of this process affords it unique advantages. For example, the process is capable of both machining and finishing simultaneously, as the lack of heat or contact removes the possibility of any surface irregularities associated with other processes, such as recast layers or burrs.
The lack of heat or contact also allows the process to largely eliminate tool wear. PECM is therefore ideal for machining high-volume parts and identical features in tandem, with a single, custom cathode.
PECM is primarily used as a machining process for complex metal components for the energy, aerospace, and medical device industries, and generally machines a workpiece that begins as bar stock or a near-net shape. However, the process can also be used as a secondary machining or postprocessing operation on additive components, and its unique capabilities can be especially advantageous for additive medical implants.
AM and Osseointegration for Spinal Cages
AM’s ability to develop customized parts specific to each patient can vastly improve safety, patient mobility and comfort. Let’s review a quick example with spinal cages, a type of orthopedic implant that can replace one or more vertebra.
One of the most important design considerations for spinal cages used in, for instance, lumbar spinal surgery, is to avoid implant subsidence, a sort of fatigue wear over time. Implant subsidence in an uneven or conventionally machined spinal cage can cause the cage to partially penetrate the vertebral endplate, which can impact the structural integrity of the implant, reduce patient mobility, and cause significant pain.
However, with modern additive technology, engineers and surgeons can produce a part that simultaneously maximizes the contact area between the implant and the vertebral endplate (uniformly distributing the load), while avoiding too large of an implant that would impair patient mobility. A Canadian study from 2022 provided evidence of this point, evaluating the respective load distribution of custom AM and conventionally-machined spinal cages–ultimately finding that the custom-manufactured cages that match the anatomical features of the patient had better functionality, durability, and osseointegration.
Despite these benefits, however, AM has inherent limitations which may disrupt both the economics of manufacturing these parts, as well as their functionality—and many of these limitations are centered around surface quality.
Surface Quality and Implants
Surface quality is crucial for the functionality and safety of orthopedic implants (as well as most other medical devices). For one, high surface quality is essential for the safety and durability of orthopedic implants involving friction, such as the “ball-and-socket” feature on the acetabular cup on a hip arthroplasty device. Not only does high surface quality reduce friction which may cause patient discomfort or limit the lifespan of the components, but materials with potentially cytotoxic ions (which we discussed earlier) are less likely to release these particles if the surface quality is smoother.
Good surface quality is also safer for the patient from an anti-bacterial perspective. Staphylococcus aureus (Staph infection), is the most common cause of periprosthetic infections by adhering itself to the implant in a ‘biofilm’. A primary way to avoid this biofilm adhesion is with superfinished surfaces across the implant (osseointegrative surfaces are, expectedly, more susceptible to biofilm; surgeons may put an antibacterial adhesive on the osseointegrative surface of the implant to ensure the bone adheres itself to the surface first).
AM’s Surface Quality Limitations
Unfortunately, modern additive technology has important limitations; it cannot produce super-finished surface qualities on most medical device components without a secondary machining process. Some causes of surface irregularities may include the layer lines themselves, re-melted material, support structure remnants, or powder particles. Furthermore, most AM processes cannot achieve adequate resolution on some features, such as downskin surfaces.
These limitations are further exacerbated when metal additive manufacturers utilize larger powders, faster laser scan strategies, thicker layer lines, or more aggressive re-coater methods to reduce costs for high-volume part production.
Limitations can also be specific to the part, such as with the spinal cages used in lumbar interbody fusion surgery mentioned earlier. While certain aspects of the cage must have a porous, osseointegrative surface, other aspects must have smoother surface quality, and these combined features may be difficult for AM processes to produce in higher part volumes.
PECM’s Fix
Fortunately, PECM may be capable of alleviating many of these limitations, acting as both a secondary machining and finishing operation for high-volume metal-AM implants, such as spinal fusion cages, hip arthroplasty devices, and others.
First, PECM can act as a secondary machining operation that can allow additive parts to achieve improved resolution—most notably on difficult features, such as thin walls and downskin surfaces. As most metal additive processes can only achieve a minimum wall thickness of around 0.3 – 0.5 mm (0.012 – 0.020 inch), a custom PECM tool can easily reduce the wall thickness to <100 µm (0.004 inch).
Furthermore, according to data from Voxel Innovations, PECM can improve the surface quality of metal 3D printed parts from 5 – 10 µm (196 – 393 µin) Ra to less than 0.5 µm (19.6 µin), even to 0.1 µm (3.9 µin) Ra. PECM is also generally quicker than conventional processes, and can replicate these thin-walled, higher-resolution features potentially tens of thousands of times without incurring tool wear.
As the process prioritizes electrical conductivity of a given material over its hardness, PECM is capable of machining complex features in tough materials commonly used in metal additive implants, such as Cobalt-Chrome alloys and titanium alloys, including Ti64.
PECM can achieve these high-resolution, high-surface quality features because there are no burrs, recast layers or surface irregularities involved in the process, and it is capable of both machining and finishing simultaneously, saving manufacturers time.
PECM could also utilize a custom cathode to selectively finish certain aspects of an additively manufactured medical part. While PECM could not replicate porous, osseointegrative surfaces on the vertebral-facing areas of a spinal cage, for instance, it could both selectively finish the side features and improve part resolution on downskin surfaces in higher part volumes.
Despite the exponential growth and popularity of metal additive processes, and its increased presence in the medical device market, manufacturers should be wary of its limitations. Therefore, they should consider an alternative postprocessing technology that can complement the strengths of AM and diminish its disadvantages. Pulsed electrochemical machining (PECM) may be capable tool by improving part resolution and creating superfinished surfaces in high part volumes.
About the author
Kirk Gino Abolafia is the technical marketing manager of Voxel Innovations in Raleigh, North Carolina, United States. For additional content on electrochemical machining, see Voxel’s education portal.
This article has first been published in the March/April edition of 3D ADEPT Mag.
Remember, you can post job opportunities in the AM Industry on 3D ADEPT Media free of charge or look for a job via our job board. Make sure to follow us on our social networks and subscribe to our weekly newsletter: Facebook, Twitter, LinkedIn & Instagram ! If you want to be featured in the next issue of our digital magazine or if you hear a story that needs to be heard, make sure you send it to contact@3dadept.com