Whenever one reads an announcement that highlights 3D printed organs, one can easily be tempted to think that these organs can already be implanted within a human body but reality shows that there is a large gap between the capabilities and limitations encountered with 3D bioprinting.
3D bioprinting is an emerging technology in which biomaterials or biomaterials combined with cells are deposited in predefined patterns, layer-by-layer using a bottom-up assembly approach to create 3D constructs that are functional 3D tissues.
This type of additive manufacturing is truly one of its kind. Fascinating for many reasons, especially for the promises it holds for transplant organs, food industry, and its ability to act as a replacement for animals in tests of cosmetic, chemical, and pharmaceutical products, 3D bioprinting could produce anything from bone tissue and blood vessels to living tissues.
“One of the ultimate goals of 3D bioprinting is to develop a viable solution that will be able to realize 3D functional complex organs but there is more than that. Research around 3D bioprinting has evolved and has started to move to industrial-based applications. One of the clear goals of 3D bioprinting is organ and tissue replacement. The other one is to create complex cell discoveries for making better medicine and quicker”, Dr. Simon Mackenzie, CEO of REGENHU told 3D ADEPT Media.
Yet, the reality, as per the words of Stephen Gray, cofounder of Ourobionics, is that “we should arrive to a solution where we merge human tissue with bioelectronics and biosensors to improve or to try to create models for drug discovery. Ultimately, we should be able to develop human electronics that could be used for implants or other medical devices. Realistically, printing an organ is a topic that we could [objectively] discuss in 10+ years, but exploring human tissue options with bioelectronics and sensors is something we can do now; it’s something that can lead to tangible improvements across segments such as drug discovery.”
“This means, the first area of focus right now is outside the body and the second stage will be replacing [or transferring] the applications inside the body” John Zandbergen, CEO of Ourobionics adds.
In fine, the significant advancements that occurred over the last decade gave a certain legitimacy to 3D bioprinting.
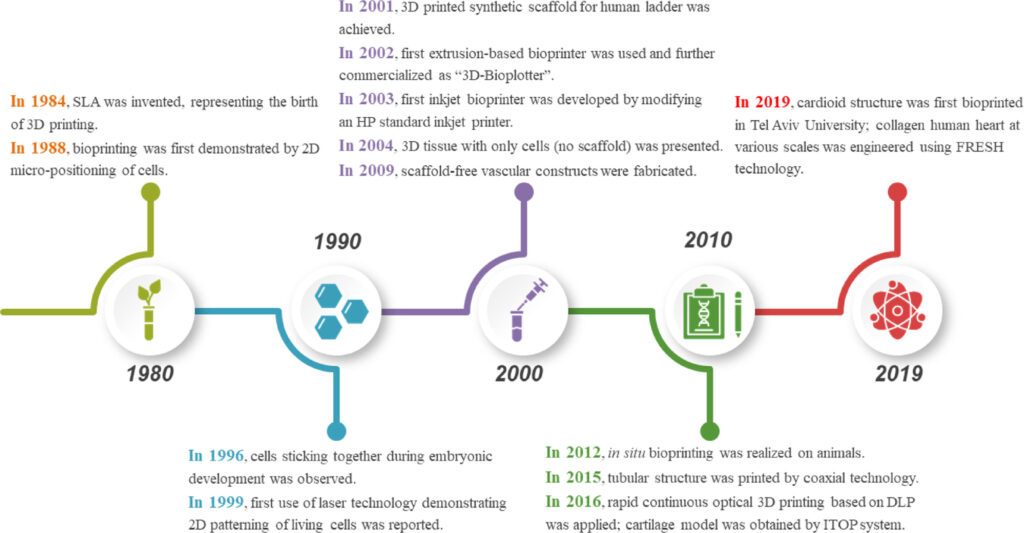
While that decade was one of awareness, we are currently living in an era where the main stakeholders strive for actions to advance this technology that is still described as “futuristic”. Where is the current market of 3D bioprinting headed? What are the current limitations of these technologies and what should be the next area of focus? These are some of the questions that this exclusive feature ambitions to address.
How does 3D bioprinting work?
When we look at 3D bioprinting processes, at how 3D bioprinting is defined and described, it’s easy to think that 3D bioprinting has benefited from several technologies such as tissue engineering, synthetic biology, micro/nanofabrication, and bioprocessing biomaterial production. Surprisingly, amid the most mentioned technologies, 3D printing does not often make that list, yet the technology should be on the top of this list.
The truth is, “the 3D printing world solved a lot of issues; we have learned [and we continue to learn] a lot from these companies. They may think it’s not their path, but they are wrong. We are just many years behind them”, Mackenzie outlines.
One of the first lessons the 3D printing world inspired, is a manufacturing process that follows the steps you probably already know: preparation (3D imaging, 3D modeling, Bioink preparation), printing process, post-bioprinting (cross-linking, maturation).
It all starts with a model of a structure that can come from anywhere – a CT or MRI scan, a computer-generated design (CAD) program, or a file downloaded from the internet. Once the 3D model file is fed into a slicer, the latter generates a series of thin layers, or slices, which form the shape of the original model when stacked vertically. The slices are thereafter transformed into path data, stored as a G-code file, which can be sent to a 3D bioprinter for printing. The constructed tissue can thereafter be post-processed in a bioreactor to recreate the required in vivo environment to maintain tissue viability during the maturation period.
Needless to say, variations in these stages may occur depending on the type of 3D bioprinting process the operator leverages and as seen with conventional additive manufacturing processes, there are also several types of 3D bioprinting processes.
Currently, scientists seem to agree on these four main categories: Microextrusion 3D bioprinting, Inkjet 3D bioprinting, Laser-assisted 3D bioprinting (LAB) and Stereolithography-based bioprinting (SLB).
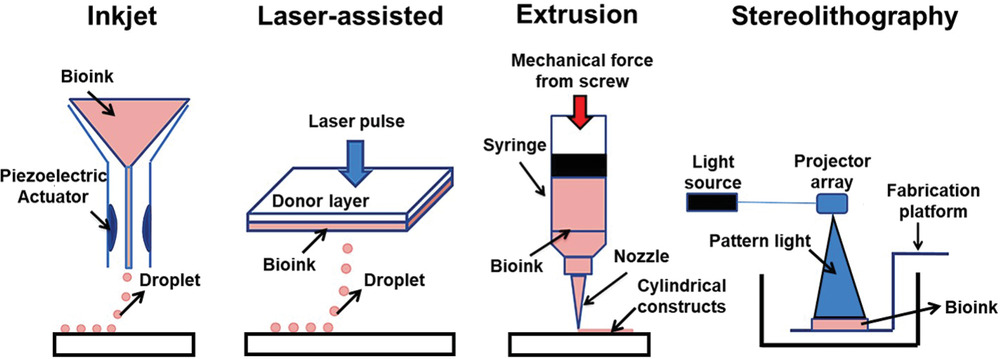
Each 3D bioprinting process comes with its share of advantages and limitations:
Bioprinting process | Description | Advantages | Limitations |
Microextrusion 3D bioprinting | Continuous dispensing of the bioink through a nozzle that is driven by a pneumatic or mechanical (piston or screw-driven) method and controlled by a computerized robotic arm. | Possibility to print high-viscosity bioinks by adjusting the driving pressure; Possibility to print tissues with very high cell densities and scaffold-free bioink; Delivers good structural integrity due to the continuous deposition of filaments | The pressure-driven dispensing results in high shear stress on the cells; which dramatically affects the cell viability; Limited resolution: inability to construct a microcapillary network. |
Inkjet 3D printing | Droplets of cell-containing bioink (each contains 10000-30000 cells) is formed through a non-contact nozzle | Non-contact based which reduces the chance of contamination; Â Possibility to integrate multi-printing heads for heterogeneous tissue structures; Fabrication of a vasculature-like structure; High-speed printing | Non-uniform droplet size; Requires bioink with low viscosity (<0.1 Pa s-1) |
Laser-assisted 3D bioprinting (LAB) | A focused laser pulse creates a bubble and shock waves that force cells to transfer toward the collector substrate. The step is repeatedly performed to create predesigned 3D structures | High precision and resolution for the printed structures which make it possible for bioprinting of micro-patterned peptides, DNA, and cells with single-cell resolution; Ability to print tissues with very high cell densities; No viscosity limitations. | The heat generated from laser energy may affect the cell viability. |
Stereolithography-based bioprinting (SLB) | UV light or laser is directed in a pattern over a photopolymerizable polymer or bioink that leads to cross-linking of the polymers into a hardened layer to form a 3D tissue | High resolution; no clogging during the printing process | Needs an intense radiation for the cross-linking; Slow process |
This table is based on the research “3D Bioprinting at the Frontier of Regenerative Medicine, Pharmaceutical, and Food Industries.”
Experts agree on the fact that microextrusion 3D bioprinting and Inkjet 3D bioprinting are the most popular processes – microextrusion being the easiest one to fabricate. Each of these processes often include a wide range of sub-processes that have their own specifications. Not to mention that the new emerging processes that have been developed during the recent years have not found a defined category yet. Some of them are categorized as hybrid technologies since they rely on multiple technology innovations. They include for instance, 3D Electrohydrodynamic Jet Bioprinting (which merges electric, magnetic, & microfluidic 3D biofabrication technology with cyborganic technology) and Melt Electro Writing. Other technologies that may not have a direct link with the aforementioned processes include magnetic 3D cell culture, acoustic assembly, micro needle array or freeform reversible embedding of suspended hydrogels.
“Those other processes that are not often found in the main categories are much more niche technologies. They are often combined with extrusion and inkjet to create better processes”, the CEO of REGENHU explains.
At the end of the day, most of these processes (if not all of them) seem to be trying to solve a pivotal issue during the printing process:
“One of the main problems with 3D bioprinting is that everything gets damage during the process. In order to create human tissue, you need something that keeps the cells alive otherwise they don’t form the tissue you are looking for. With extrusion bioprinting you can get some basic structures, but you can’t go to the next level”, Stephen Gray, cofounder of Ourobionics told 3D ADEPT Media.
Another aspect we should take into account is the level of complexity, Zandbergen says. “You cannot create different kinds of complexity with the same tissue. Each tissue presents its array of limitations depending on its purpose. The level of complexity you are able to create inside the 3D structure determines the level of functionality of the tissue you are making. Don’t expect a huge level of complexity when it comes to the depth of the tissue when using basic bioprinting processes”, he points out.
So, what are some of the key criteria that may lead to the choice of one process over another?
Let’s remember that given the relatively “nascent” nature of this field of activity, most of the criteria that may help scientists to go for one process over another often depends on several experiences gathered from different users. In this specific case, our interviews and research reveal that a few criteria tend to come back in the decision-making process of scientists.
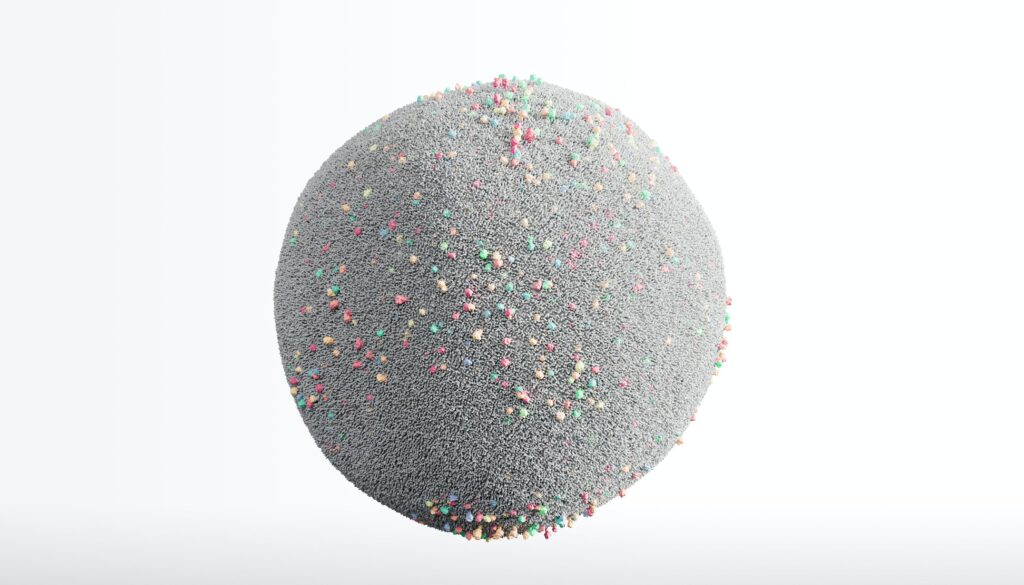
The first one is cells. As speakers from Ourobionics said, the goal here is to keep the cells alive while working on them. Tissue printing requires a large number of cells. “It’s a very crucial problem because you cannot create a human tissue if everything is dying in the process. One of the recent improvements in this area has been to add a volumetric principle to the printing process of some technologies. Light-based technologies are also getting to a level where they are slightly better but the problem here remains inside the resin – which in my opinion – cannot deliver a functional organ”, Zandbergen lays emphasis on.
Another criteria is speed/time. Based on Mackenzie’s explanation,we quickly understand that it is also linked to cells, as he explains that when they do not have the ideal speed, some technologies can destroy cells during the printing process. The thing is, created structures can change their morphology in time once they are in contact with certain stimuli (water, heat, and light) but the embedded cells can also proliferate, migrate, and differentiate along the time, forming more mature tissues with higher resemblances with the native tissue.
No matter what number of criteria we will mention, one that should never be underestimated is the final purpose. At the end of the day, each 3D bioprinting process has its share of pros and cons – and some work well for certain purposes. Extrusion-based desktop printers that integrate small syringes for example, can only be used to deposit bioinks and hydrogels with incredible precision (up to 1 μm) while machines that combine different bioprinting deposition methods in one single technology can be dedicated to producing artificial human tissues for research and development.
Applications and areas for improvement
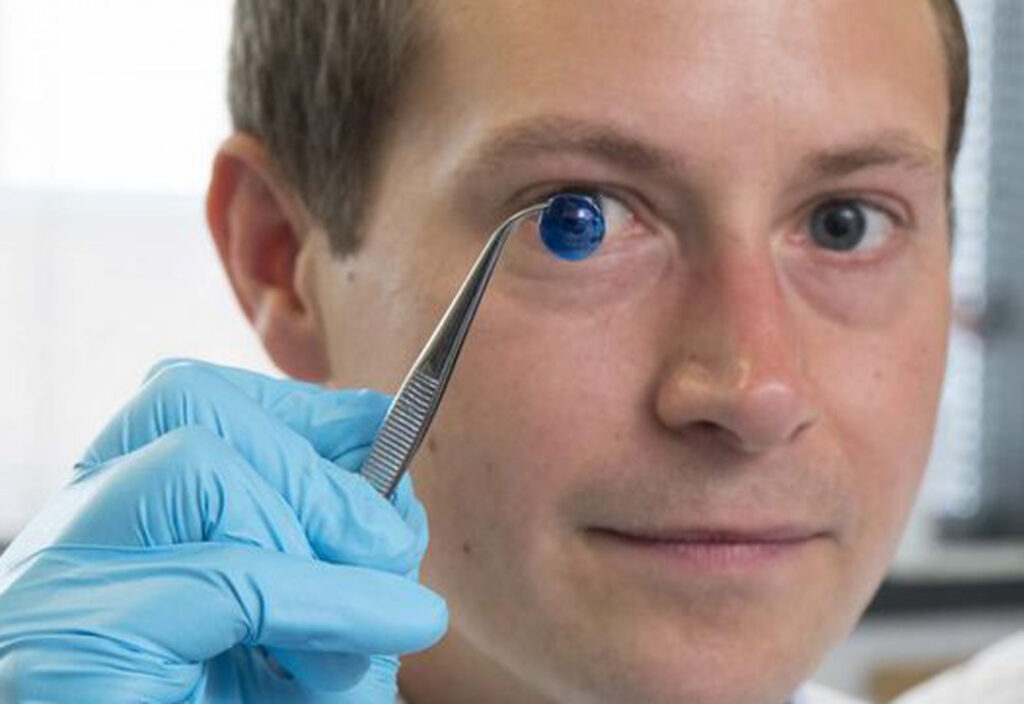
“While many technologies touch upon organ and tissue replacement, we are still very far away from commercial applications. On the current market, the cell biology side is gaining momentum, especially for complex cell models as they ambition to reduce the need to use animals for testing products. Applications that are closed to market include the printing of beta cells for diabetes”, Mackenzie’s points out.
Bioprinting paves the way for creating biomimetic structures and environment that support in vivo-like cell–cell and cell–matrix interactions with high-resolution vascularized tissue. In this regard, Bioprinted tissue would represent powerful tools to provide physiologically relevant in vitro human organ models for drug toxicity assays and disease modeling that faithfully reproduces the complex human’s key physiological aspects.
Another promising application of the future is In situ Bioprinting, which might enable bi-layered skin to be printed directly into a wound.
Lastly, applications that are already commercialized can be seen in the food industry with cultivated meat. Alternative meat is currently trendy in the 3D printing industry as people are increasingly aware of the ethical and environmental issues surrounding conventional animal husbandry. A few companies like MeaTech or Redefine Meat that have dedicated their core business to this market have already started commercializing their meat alternative in select restaurants across Europe.
However, there are a number of limitations that remain to address to help the market move forward efficiently:
Areas for improvement | Why? |
Complexity | There is a complex process to bring together the instruments and the cells. And set out protocols can take a long time. |
Biomaterials | Current available printable materials are not capable of fully mimicking the native ECM compositions to support the cellular structure. Therefore, it is crucial to develop new printable biomaterials that can be printed together with live cells and possess adequate mechanical properties for cell handling. |
Biomaterials | A lot of them are still not optimal. The hardware is capable of much more than the biomaterials are capable today. Â |
Software | Software is quite complex and there is no area of control that we might see for complex structures. |
Files | Everyone uses their own files. There is an urgent need of standardization. |
Price | A technology with such ambitious goals should be accessible in order to achieve them. Tissue engineering is a larger market and the lack of accessibility to hardware does not enable some institutes to advance this technology. They only have access to basic extrusion for education purpose which is not the right route to go to address the challenges on the road. |
Table: Limitations outlined based on interviews with REGENHU and Ourobionics
Concluding thoughts
Our experience in the industry taught us one lesson: there are many technologies that promise a lot and deliver little. That’s why we do not usually highlight or discuss a technology that is not commercialized yet or that has not demonstrated viable commercial applications. Yet most 3D bioprinting technologies are currently being used for research purpose – for now.
When you know that each day, 17 people die waiting for a life-saving organ transplant and a new name is added to the transplant waiting list every 9 minutes. Currently, there are more than 100,000 people waiting for a second chance; not to mention those who experience chronic problems due to the long-term damaging effects of post-transplant immunosuppression.
When you know that, and you realize that 3D bioprinting can be the alternative solution these people are waiting for, when you know that the technology can do more than that, you can’t help but highlight the areas for improvement that should be the next focus of (3D bioprinting) companies – hoping that this might urge some of them to explore new solutions and others to continue the battle.
 This dossier has first been published in the May/June edition of 3D ADEPT Mag.
Resources and contributors:
REGENHU started in 2007 with a goal to create and develop bioprinting technologies that will positively impact many medical fields. The company’s R-GEN 100 and the R-GEN 200 consolidate 14 years of bioprinting technology and development based on incorporating industry knowledge, integrating user feedback, and responding to market requests.
 Ourobionics is a startup that merges advanced electric, magnetic, & microfluidic 3D biofabrication technology with novel cyborganic technology to create high throughput tissues & organs with embedded bioelectronics, biosensors, and therapeutic agents. The company ambitions to enable next generation applications in regenerative medicine, medical devices, & human-machine interfaces.
3D Bioprinting at the Frontier of Regenerative Medicine, Pharmaceutical, and Food Industries, Front. Med. Technol., 28 January 2021 |Â https://doi.org/10.3389/fmedt.2020.607648
Development of 3D bioprinting: From printing methods to biomedical applications, research, Asian Journal of Pharmaceutical Sciences
3D Bioprinting of Lignocellulosic Biomaterials, https://doi.org/10.1002/adhm.202001472
Remember, you can post job opportunities in the AM Industry on 3D ADEPT Media free of charge or look for a job via our job board. Make sure to follow us on our social networks and subscribe to our weekly newsletter : Facebook, Twitter, LinkedIn & Instagram ! If you want to be featured in the next issue of our digital magazine or if you hear a story that needs to be heard, make sure you send it to contact@3dadept.com